This month's Accretionary Wedge #61 topic, hosted by
GeoMika, is asking
what exactly everyone does in the real world as a geoscientist. When students ask, "what can I do with this degree?", it's difficult to answer, because there are so many ways to be a geoscientist. For this month's post, we're answering that question with "well, you can do a lot of things - but this is what I do". So, I will tell you about what I do as a sourcewater protection hydrologist.
Specifically, I work for the State of Minnesota, tasked with protecting potable groundwater throughout the state. This is my first "real" career job out of school, so everything I've done is a new experience - especially figuring out which aspects of my education I actually use and which I don't. I'll point them out as I go. I have a basic undergraduate geology degree (obtained while in Minnesota), and a master's degree focusing on karst hydrology and GIS (from down in Missouri).
To summarize, my hydrological duties consist mostly of officework with a minor component of fieldwork. I will focus on the officework for this post.
My main ongoing task is performing and writing groundwater protection reports for the many small cities throughout the state. Each city that has a municipal drinking water system gets one of these reports every few years (to account for changing conditions or updated information) describing the flow of groundwater towards their wells. By knowing where the groundwater is flowing from, an
area on the land surface can be drawn to protect the water they drink, called the wellhead protection area.
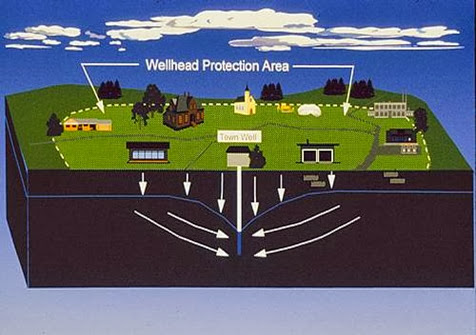 |
Illustration of a Wellhead Protection Area, showing where on the land surface recharges groundwater for a well. |
This is accomplished through groundwater modeling. There are many types of methods and software to do this. As the saying goes in science, "all models are wrong, but some are more useful than others". To run these models, I need varying amounts of the following information: pumping rates of wells, aquifer properties (thickness, material, flow boundaries) and hydrologic properties (conductivity, water levels, flow gradient). In other words, data mining to start with followed by looking at all the important data to describe the local hydrology and geology. Luckily, our state has an extensive
well GIS database, with each well providing important information, such as water level and stratigraphy from driller's logs.
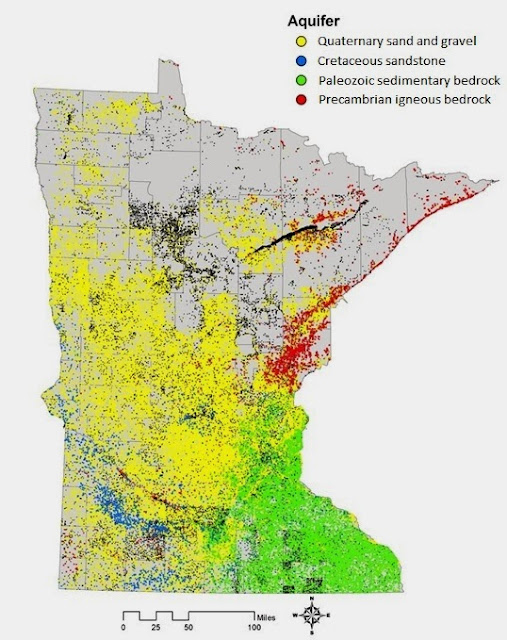 |
There are over 260,000 wells in Minnesota's County Well Index, each with much useful information, such as aquifer type. No shortage of data here for the GIS-savvy. |
One of the early steps is producing a basic groundwater flow field near the area I'm studying . Almost every geology student will perform something similar to this in a lab - usually hand contouring water levels on paper. The same principles apply here - groundwater flows from high to low water level elevations and perpendicular to the contours. A rough version of this can be created in ArcMap by selecting the nearby same-aquifer wells and then using the geostatistical tools on the recorded water levels. This requires a basic understanding of hydrology and handiness with ArcMap (definition queries, geostatistical tools, editing).
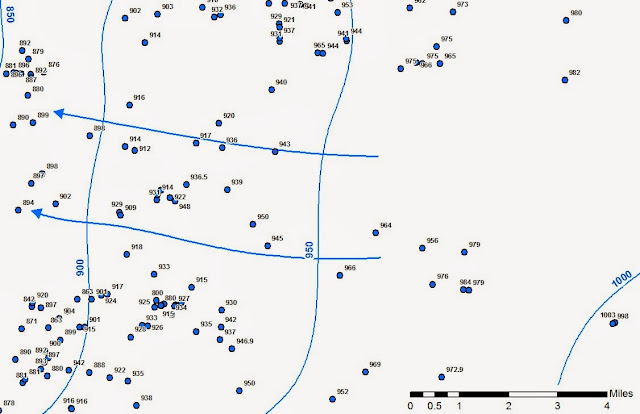 |
Local groundwater flow field produced from contouring well water elevations using geostatistical tools in ArcMap. |
My favorite part of this process is describing the local geology through cross-sections from well logs and published materials. There is always plenty of information to start with - chances are good that either the well driller or the city already know what kind of aquifer they are drawing from (glacial sands, igneous bedrock, sedimentary bedrock, etc.), so I know which maps to refer to. One or two cross-sections are planned to best describe the subsurface.
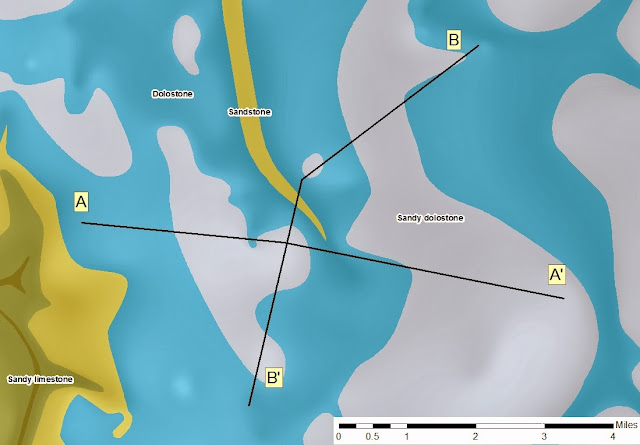 |
Shaded bedrock map with cross-sections A and B. The line A-A' represents the cross-section below. |
There is a lot of room for interpretation here, as well logs contain the information recorded while drilling the well, and drillers sometimes have their own unique vocabulary and varying attention to detail (from the supremely uninformative "rock" to redundant "clayey-clay"). It's also where you get to be artistic. A crude cross-section can be made from the well logs alone, but is better refined by then referring to any published geologic maps or shapefiles. Everything but the drawn geologic units below is quickly created using the GIS tools and well data available to us (the vertical lines are driller's logs with colors representing different units or rock/sediment types. It even puts in the elevation lines and topography - it's almost too easy!).
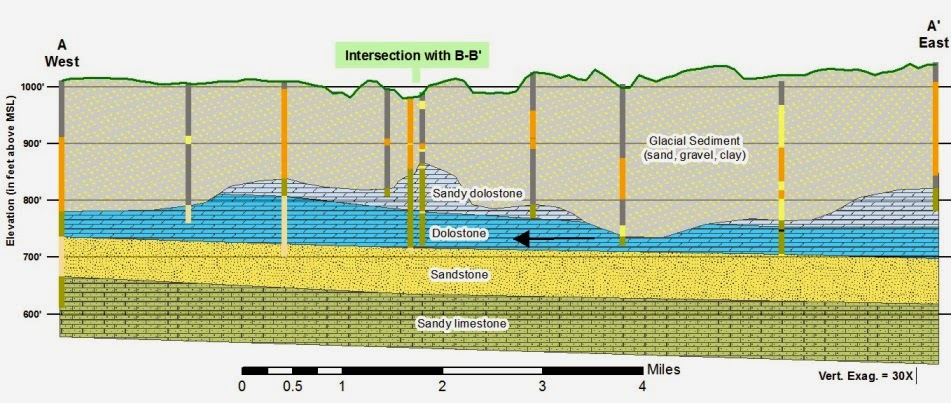 |
A geologic cross-section drawn from driller's logs. The
aquifer used in this region is the combined dolostone (blue) and
sandstone (yellow) units. Not the irregular bedrock topography due to
glaciation and pre-glacial stream valleys. |
By combining the flow field with the cross-sections, I get a basic understanding of the local hydrogeology. For this site, the wells draw from both of the dolostone units (light and dark blue) and the sandstone (yellow). Both units are buried under nearly 200 feet of Quaternary glacial sediment (which includes lots of clay), so the aquifer is well protected from rapid surface recharge - a good thing. I always like seeing the buried valleys in the bedrock - remnants of the last Ice Age. This requires handiness with ArcMap (again), basic stratigraphic principles, and some artistic and interpretive license (there is no standard to how these are made - some hydrologists settle for crude, choppy, black-and-white affairs. I take pride in my color schemes).
The fact that the well above draws from two types of sedimentary bedrock; a dolostone and a sandstone, is important, as groundwater flows differently in these different rock types. Water flows between the sand grains in the sandstone unit, while water flows through fractures in the dolostone. Both bedrock types require different modeling techniques, so more than one model is run for the well to account for this.
After identifying aquifer thickness, type, hydraulic conductivity (from pumping tests, or calculated in other ways), and pumping rates from wells, I can insert this information into one of many different groundwater modeling programs. These programs attempt to emulate the local flow conditions by incorporating the regional groundwater flow lines with gradients produced from pumping wells (like how a black hole warps gravity, drawing nearby things toward them).
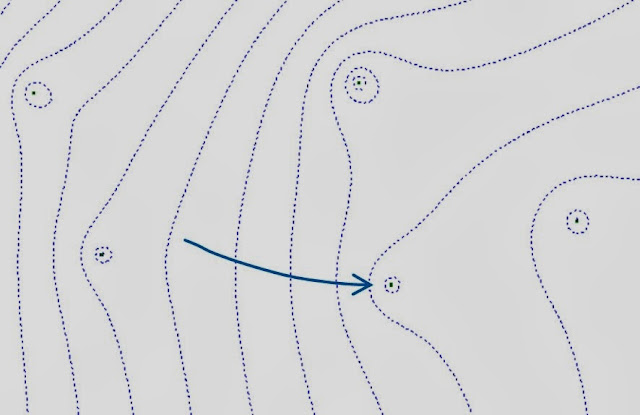 |
A groundwater flow field model incorporating pumping information from nearby wells. Note how the wells warp the regional flow lines. |
From there, I just specify which well I want to draw some flow pathlines to, and the travel time (say, 15 years). This will work backwards to show the extent of particle lines feeding that well for that amount of time (everything in that area will reach the well in 15 years).
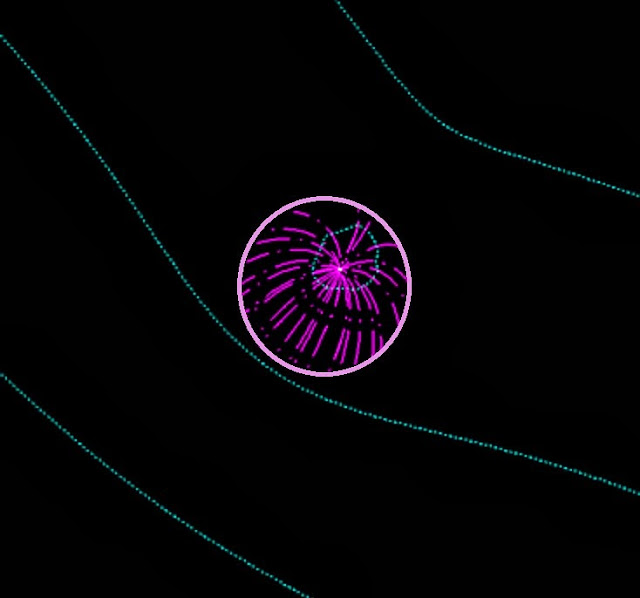 |
Simple model of particle lines (purple) flowing to a well for a given time. Groundwater flow is from bottom left to top right. |
The better I understand the local geology and hydrology, the better the resulting model. I had no real background in groundwater modeling before starting here, but most of these programs are easy to learn, though difficult to master. A willingness to learn is the basic requirement for this part of my job, although some knowledge of numerical hydrology will go a long way. The end result from this modeling is the Wellhead Protection Area (see
illustration towards the top of the post) which is converted into a
Drinking Water Source Management Area (see below, made by enclosing the Wellhead Protection Area in with notable surface features, such as roads or political boundaries). This information is available to the public, and you can even look up the groundwater protection area for your city
here.
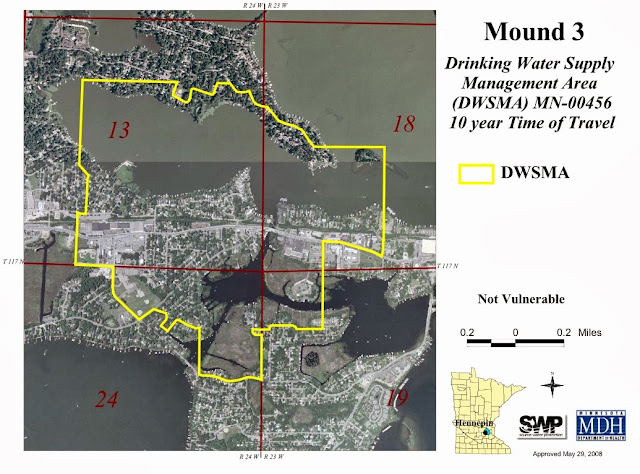 |
Drinking Water Supply Management Area for Mound, Minnesota. The aquifer is deemed Not Vulnerable based on water chemistry and geologic information. |
In order to do what I do on a daily basis, I regularly rely on what I learned in hydrology, structural geology, stratigraphy, and GIS courses; some fairly basic coursework. Each of these reports is like a mini research project, so having performed an undergraduate project and a master's thesis is important. But aside from those points, I there was one class which have especially helped me be better at my job, while not being necessary to doing it.
One of the most useful geology classes I ever took was one of my first. It was called Minnesota's Rocks and Waters - a geology class for non-majors. I took it along with the Introductory to Geology class, just as a filler. It was one of those large classes that people took to get a science credit. It was a fairly in-depth look at all of Minnesota's unique geological regions, and what got me inspired to pursue geology as a career.
Despite being a low level class, it laid down a foundation which allowed me to have a head start on all of the geology trips I would go on throughout the state. It woke me up to the world of geology by making it local - to learn about why there are igneous and metamorphic rocks here, or why there are sandstones and limestones there, and why there are rolling hills of glacial clay and sand throughout most of the state - information I still use today. Whenever I start a new groundwater protection report in another part of the state, I get to remember what I learned in that first class years ago, and why I fell in love with geology. It didn't introduce me to any advanced geologic topics, but it was informative, and I get to use that information daily, which makes it useful. I always keep the text from that class nearby.